Constraining the formation conditions of modern pisoids at Ore Lake, Michigan
DOI:
https://doi.org/10.57035/journals/sdk.2023.e11.1179Keywords:
Pisoids, Stable isotopes, Lacustrine, Carbonate, Modern analogueAbstract
Large concentrically laminated carbonate grains (here referred to as pisoids) have been observed sporadically throughout the geological record and in modern environments. Explanations for how these grains form have varied widely in different settings, although microbial effects are often involved. In Ore Lake, a ~1 km2 flow-through lake in southeast Michigan, one to four centimeter oblong calcite pisoids are observed in both lake bottom shallows and mounded as a small spit near the primary outflow. In section mm-scale light and more porous along with and dark and more dense concentric laminations are apparent. Here we use field observations, petrography, water chemistry, and stable isotopes to understand their formation. Measurements of pisoid calcite δ18O and lake water δ18O indicate that precipitation occurs in waters between roughly 19 – 28°C. These warm temperatures imply that pisoid growth happens almost entirely within the summer, contrary to prior work that suggested wintertime precipitation was important. Pisoid δ18O values largely overlap with coexisting lake bivalve values, suggesting that pisoid precipitation is in equilibrium. In contrast, pisoid δ13C is as much as 8 ‰ more positive than bivalve δ13C due to photosynthetic effects. We propose that the laminations in these pisoids arise from different rates of formation within the warm months, rather than large seasonal differences. A decline in lake alkalinity beginning in late spring likely coincides with more rapid growth, with slower growth mediated by cyanobacteria continuing through the summer. This range of observations enables the use of Ore Lake as a potential model for understanding pisoid formation throughout the geological record.
Downloads
Lay Summary
Here, we investigate how unique, concentrically layered grains (“pisoids”) are forming in a small inland lake in Michigan, USA. We use field observations, thin sections, and measurements of grain and water composition to learn about their formation. We find that the pisoids form in close association with local cyanobacteria during the warm months, which are likely moved around as they grow. Studies that examine how pisoliths form today can inform how we interpret them in ancient geological deposits.
1. Introduction
Carbonate precipitation in association with cyanobacteria is common in ancient and modern settings, but the character of the precipitate and the precise details of how it forms are highly variable (Pedley, 1990; Benzerara et al., 2014; Chafetz et al., 2018). The morphology of microbial carbonates can differ greatly; such structures include tufa, travertine, stromatolites, oncoids, spherulites, microbialites, and pisoids or pisoliths (Chafetz & Meredith,1983; Folk and Chafetz, 1983; Riding, 1983; Rivera & Sumner, 2014; Chafetz 2018). Settings where cyanobacteria-driven precipitation occurs are thought to include nearly all places where carbonates form, including hot springs and geysers (Chafetz, 2018), shallow marine environments (Reid et al., 2000), and freshwater environments such as lakes (Andrews & Trewin, 2014; Chagas et al., 2016) and streams (Merz-Preiß & Riding, 1999; Andrews, 2006), from the modern and Holocene through the Archean (e.g., Rivera & Sumner, 2014).
Figure 1. Map of water and carbonate sample locations within Ore Lake.
Concentrically laminated carbonates (including microbialites) in particular have attracted interest for decades (e. g., Irion & Müller, 1968; Dunham, 1969; Dahanayake, 1977; Risacher & Eugster, 1979). Broadly defined, these concentric structures larger than ooids also have been described from modern settings and throughout the geologic record (e.g., Monty & Mas, 1981; Maliva, 2000; Rivera & Sumner, 2014). Concentric laminations are known to form from both biotic and abiotic factors. In some cases, vadose zone precipitation (Dunham, 1969) and/or hypersaline precipitation (Esteban & Prey, 1983) are thought to create concentric laminations abiotically. However, cyanobacteria likely play a role in most concentric carbonate formation processes (e.g., Barth & Chafetz, 2015; Mors et al., 2022). For example, bacteria can help to instigate precipitation that proceeds in part abiotically (Chafetz & Meredith, 1983; Barth & Chafetz, 2015), or play a role in precipitation throughout the growth of concentric structures (Chafetz et al., 2018). Indeed, the presence of amino acids and extracellular polymeric substances (even when the organisms were no longer living), are enough to induce carbonate precipitation in concentric patterns (Braissant et al., 2007). The common assumption of microbially induced precipitation, in general, is that given the ‘right’ conditions (e.g., particular saturation states, temperatures, and alkalinity), cyanobacteria can promote carbonate precipitation by altering local water chemistry through photosynthetic processes (Merz-Preiß & Riding, 1999; Braissant et al., 2007; Müller et al., 2016; Chafetz et al., 2017; Turchyn, 2021; Shiraishi et al., 2022).
This paper focuses on freshwater pisoids in a humid continental setting at Ore Lake, Michigan, USA (Figure 1), an inland, temperate marl lake (Binkley et al., 1980). The definitions of ‘pisoids’ differ in the literature; here we use the term to describe oblong to circular, concentrically banded, tufa-like structures one to four centimeters in diameter (Figure 2). At Ore Lake, these calcareous forms have been found to have both skeletal (gastropods and bivalves) and non-skeletal nuclei. Nearly all samples display concentric banding around the center. The samples observed in this study are all thought to be modern. Ore Lake has long been noted for carbonate deposition in the form of marl (Pollock, 1919), pisoids (Jones & Wilkinson, 1978), and carbonate-cemented beachrock (Binkley et al., 1980). Cyanobacteria (previously known as ‘blue-green algae’) are seasonally abundant in Ore Lake (Parker & Kohlhepp, 2017) and are understood to play a role in local carbonate formation for over 100 years (Pollock, 1919).
Figure 2. A range of complete pisoids that were sampled from Ore Lake. Scale bar in millimeters.
Jones & Wilkinson (1978) concluded that the Ore Lake pisoids likely remained in place during precipitation, based on the general observation of flattened bases of their samples. They determined that this flattening represented an area of the pisoid that remained in contact with the lake bottom sediment. While precipitation favors the side of the structure that is most available for photosynthesis, they noted that precipitation must occur on all sides of the pisoids to obtain the concentric banding observed. Jones & Wilkinson (1978) also noted that clear dark and light banding within the pisoids was due to variations in porosity (Figure 3), with porous layers containing more filamental structures. They concluded that this difference in layer composition indicated year-round precipitation, with photosynthesis only contributing to carbonate precipitation during warmer months and a nearly equivalent amount of abiotic precipitation occurring during the winter (Jones & Wilkinson, 1978). In this study, we seek to reassess whether such substantial carbonate precipitation during winter months (when our observations indicate the lake surface is often partially frozen) is likely.
Figure 3. Cross-section of pisoid sample SP1, which was sub-sampled for stable isotopes (small holes). Note circular concentric layering.
This study aims to broadly refine the conditions of carbonate precipitation in Ore Lake, particularly investigating pisoid formation and the possibility of winter precipitation (perhaps abiotic). We use stable isotope data to constrain the water chemistry and temperature at the time of pisoid formation. We also assess the location of pisoid formation and transportation within the lake; field observations indicate that Ore Lake has sufficient flow for grains in shallow areas to be transported towards the lake outflow in the south (Figure 1). This study evaluates how these findings may be relevant for studies of similar concentric grains throughout the geological record.
2. Methods
2.1. Sampling
Water samples were collected from Ore Lake over a visit in October 2016, one visit in February 2017, and three visits between July and October 2021. Single-visit sampling also included the connecting Huron River, four nearby lakes (Zukey, Bass, Bishop, and Lime), South Ore Creek (which flows into Ore Lake), and one sample from a nearby park faucet located southeast of Ore Lake, which was sampled to represent local groundwater (Supplemental Figure 1). Carbonates analyzed here were collected from Ore Lake in July 2021 from the northern shore and a spit on the southern shore (Figure 1; Figure 4).
Water sampling was conducted using sterile 10mL syringes, welded syringe filters, and 5mL glass vials. These samples were collected from surface waters using a boat as well as from the shore, depending on the trip and the targeted areas. Alkalinity testing was conducted using the titration method, where total alkalinity was expressed in mg/L (Lamotte 4491-DR-01 Water Quality Testing Kit). In-the-field testing of water pH, conductivity (µs), and total dissolved solids (ppm) was conducted using a water quality meter with a +/-1% accuracy.
Figure 4. Field photo of surficial sediments on the spit at the southern end of Ore Lake (see map in Figure 1). The very coarse-grained sediment here is dominated by disarticulated quagga mussel shells, complete pisoids , and pisoid fragments.
2.2. Stable isotope methods
δ18O and δD of water samples collected in 2021 were measured at the Iowa State University Stable Isotope Lab (ISU). Older samples from 2016 and 2017 were measured at the University of Michigan (U of M). Both sample sets were measured using a Picarro L2130-i Isotopic Liquid Water Analyzer, with an autosampler and the ChemCorrect software. For ISU samples, the final three injections (of six total) were used to calculate mean isotopic values to account for memory effects. For U of M samples, the last four analyses of nine measurements were used. In both labs, USGS 47 and USGS 48 reference standards (USGS, 2023) were used for regression-based isotopic corrections and to assign the data to the appropriate isotopic scale in which at least one standard was used for every five samples. The combined uncertainty (analytical uncertainty and average correction factor) for water δ18O (δ18Owater) is ± 0.1 ‰ (VSMOW) and for δD is ± 0.5 ‰ (VSMOW).
Carbonate δ18O and δ13C of sixteen pisoids and four shells identified at genus level (threeDreissena and and one Corbicula) were measured at the U of M Stable Isotope Lab relative to internal standards and the Vienna Peedee Belemnite standard (VPDB). Four of these pisoids were subsampled in multiple places across apparent growth laminae (Figure 3). The subsamples were obtained using a handheld Dremel tool, which unfortunately was too large to enable reliably discrete sampling from pisoid layers (that is, several subsamples include some portion of a light and darker layer). The other twelve pisoids had one sample taken from the outer surface of each, which was completed after abrading away the outermost ~1 – 2 mm of carbonate and debris. The bivalves were sampled from just one location along the outside of the shell. All samples were measured for both δ18O and δ13C using a Kiel IV automated preparation device coupled to a Thermo Scientific MAT 253 mass spectrometer at the University of Michigan Stable Isotope Laboratory. The analytical precision was greater than 0.1 ‰.
Formation temperature was calculated from carbonate δ18O data using mineral-specific fractionation factors. For calcite pisoids we used Kim & O’Neil (1997). For aragonite shells we used Grossman & Ku (1986). For all samples we input a δ18Owater value of -5.6 ‰. This value is the mean of all δ18Owater measurements within Ore Lake, excluding the single sample taken during winter. The effect of changing the δ18Owater value used for calculating temperatures (including the winter δ18Owater value, for example) is discussed below. We treat these calculations as an estimate of temperature and not as an exact measurement. This is because δ18Owater certainly varies through time and within the lake (although the data suggest that lake δ18Owater values are relatively constant across summer and fall; see results).
3. Geochemical results
3.1. Field observations
We located pisoids in four locations in Ore Lake: partially buried within sediment at the northern shore near where South Ore Creek enters Ore Lake, two locations along the east side of the lake, and as part of an elevated spit at the southern end (Sample Location map). This ~60 m spit structure is made up primarily of mollusk shells, coarse carbonate sand, fragmented pisoids, and complete pisoids (Figure 4). The spit juts into the water where Ore Lake drains into the much smaller (~0.05 km2) Little Ore Lake and subsequently the Huron River to the south, helping to separate the two lakes. The composition and morphology of the spit, as well as the north-to-south current in Ore Lake, suggest that it formed from apparent changes in shoreline currents and/or wave energy at this drainage point.
The pisoids collected from the northern shore of the lake (where South Ore Creek drains into it) were located < 5 m from shore and were sampled from under approximately 25 – 75 mm of sand. The pisoids were much less abundant than in the area in and around the southern spit (Figure 1). The burial of these samples may have been due to high lake levels (and creek discharge) occurring at the time of collection. In addition, pisoids were located in two areas in the eastern part of the lake, where a shallow (< 3 m) shelf extends ~200 m from shore. These were observed and sampled in water ~2 m and ~0.75 m deep.
Sample | Location | Sampling Date | Latitude / Longitude | δ180 (VSMOW) | δD (VSMOW) | Alk. (mg/L) | pH | Cond. (µs) | TDS (ppm) |
OLWI | South Ore Creek | 7/17/2021 | 42.49770N83.80210W | -5.87 | -43.06 | 156 | no data | no data | no data |
OLW2 | Huron River | 7/17/2021 | 42.47500N83.78850W | no data | no data | 144 | no data | no data | no data |
OLW3 | Ore Lake (shoal) | 7/17/2021 | 42.47580N83.79660W | -5.75 | -43.55 | 136 | no data | no data | no data |
OLW4 | Ore Lake (middle) | 7/17/2021 | 42.47780N 83.79690W | -5.77 | -43.45 | 148 | no data | no data | no data |
BILW24 | Bishop Lake | 7/17/2021 | 42.50260N 83.84260W | -2.65 | -29.62 | 88 | no data | no data | no data |
ZLW25 | Zukey Lake | 9/4/2021 | 42.46310N 83.84690W | -4.25 | -32.8 | 124 | no data | no data | no data |
BALW26 | Bass Lake | 9/4/2021 | 42.44900N 83.85680W | -4.56 | -35.09 | 128 | no data | no data | no data |
OLW8 | South Ore Creek | 9/4/2021 | 42.49040N 83.79860W | -5.25 | -38.81 | no data | no data | no data | no data |
OLW9 | Little Ore Lake (south) | 9/4/2021 | 42.47380N 83.7969W | -5.19 | -38.79 | 144 | no data | no data | no data |
OLW10 | Well Water Sample | 10/2/2021 | 42.4752N83.7825W | no data | no data | 212 | 8.28 | 661.4 | 447.3 |
OLW11 | Little Ore Lake (middle) | 10/2/2021 | 42.47420N 83.79710W | -5.93 | -43.6 | 140 | 8.91 | 778.6 | 533.8 |
OLW12 | Ore Lake (shoal) | 10/2/2021 | 42.47590N 83.79660W | -5.52 | -41.3 | 140 | 8.93 | 777.9 | 535.1 |
OLW13 | Ore Lake (middle) | 10/2/2021 | 42.8070N83.7967W | no data | no data | 140 | 8.96 | 778.5 | 535.6 |
OLW14 | Ore Lake (north) | 10/2/2021 | 42.48550N 83.79750W | -5.62 | -41.77 | 164 | 8.8 | 780.6 | 536.5 |
OLW15 | South Ore Creek (Brighton) | 10/2/2021 | 42.53500N83.7844W | no data | no data | 168 | 8.56 | 649.4 | 449.9 |
OLW16 | Lime Lake | 10/2/2021 | 42.5273N83.8119W | no data | no data | 128 | 8.36 | 933.2 | 646.5 |
OLW17 | Ore Lake (middle) | 10/3/2016 | 42.48060N 83.79590W | -5.47 | -40.27 | no data | no data | no data | no data |
OLW18 | Little Ore Lake (middle) | 10/3/2016 | 42.4742N 83.79730W | -5.5 | -40.91 | no data | no data | no data | no data |
OLW19 | Ore Lake (south) | 10/3/2016 | 42.47600N 83.79690W | -5.65 | -41.45 | no data | no data | no data | no data |
OLW20 | Ore Lake (north) | 10/3/2016 | 42.48570N 83.79810W | -5.56 | -41.15 | no data | no data | no data | no data |
OLW21 | East Ore Creek | 10/3/2016 | 42.49000N 83.79100W | -6.92 | -47.18 | no data | no data | no data | no data |
OLW22 | South Ore Creek | 10/3/2016 | 42.4977N83.8021W | -6.45 | -44.88 | no data | no data | no data | no data |
OLW23 | Ore Lake (northwest) | 2/17/2017 | 42.48480N 83.80150W | -7.81 | -52.08 | no data | no data | no data | no data |
3.2. Alkalinity
The alkalinity of lake water indicates the availability of bicarbonate ions for uptake during photosynthesis, which in turn increases the likelihood of carbonate precipitation by cyanobacteria or algae (e.g., Merz, 1992). As far as we have observed, Ore Lake is locally unique in forming pisoids and/or significant microbialite formation. Enhanced carbonate precipitation in Ore Lake is therefore consistent with elevated alkalinity relative to nearby lakes. Our measurements of Ore Lake alkalinity range from 136 to 164 ppm CaCO3 (Table 1). Measurements from nearby Bishop, Zukey, and Bass lakes range from 88 to 126 ppm CaCO3 (Table 1). Water samples taken from South Ore Creek showed the greatest surface water alkalinity concentration, ranging from 156 – 168 ppm CaCO3. As presented in Supplemental 2, there is a decline in surface water alkalinity from the north to the south, with higher alkalinity South Ore Creek waters mixing with other sources (including groundwater) within Ore Lake (Figure 1). As such, the northernmost sample location within the lake also had the highest alkalinity within the lake. The potential relationship between spatial variability of lake surface alkalinity and pisoid formation is discussed below.
We supplement these data with historical alkalinity measurements collected by the Ore Lake Preservation Association (Fusilier, 1987, 1999) to evaluate seasonal alkalinity patterns within the lake. Figure 5 shows that Ore Lake alkalinity reaches a maxima in the spring and rapidly declines into the summer. It is likely that after reaching a late summer minima, alkalinity slowly increases throughout the fall and winter; this relationship is observed in other small lakes with abundant carbonate (Otsuki & Wetzel, 1974). This seasonal cycle probably coincides with the onset of major carbonate precipitation within the lake during the spring and summer, and reduced carbonate precipitation into the late fall and winter. No major change in lake alkalinity over time (during the 1980s, 1990s and 2020s) is observed in the summertime data. The historical alkalinity data have values that overlap and do not indicate clear change over years. Other water chemistry data (pH, conductivity, and total dissolved solids) are also reported in Table 1.
Figure 5. Historical and recent seasonal alkalinity data from Ore Lake. We collected the 2021 and 2022 data; the older data were collected by the Ore Lake Preservation Association. Note that spring (April) data are all higher than summertime samples.
3.3. Stable isotope results
Pisoid δ18O values range between -6.33 ‰ and -8.12 ‰ VPDB, with a standard deviation of 0.5 ‰. These values are broadly consistent with the bivalve shells that were sampled (mean of -6.8 ‰; Table 2). In contrast, δ13C values differ considerably between pisoid and bivalve carbonate, well exceeding any potential mineralogical effect (pisoids are calcite and the shells are composed of aragonite). Pisoid δ13C values range between -1.1 and -3.9 ‰ VPDB, with a standard deviation of 0.6 ‰, while mollusk samples range between -5.1 and -9.1 ‰, with a standard deviation of 1.6 ‰. δ18O values of water samples collected in Ore Lake during summer and fall range from -5.8 ‰ to -5.5 ‰; δD ranges from -43.6 ‰ to -40.3 ‰ (VSMOW; Table 1). A single wintertime water measurement (taken on February 17, 2017) had a δ18Owater value of -7.8 ‰ and a δD value of -52.1 ‰ (Supplemental 3).
3.4. Formation temperature estimates from carbonate δ18O
Using the mean summer and fall Ore Lake δ18Owater value of -5.6 ‰ VSMOW (see methods), calculated formation temperatures for pisoid calcite range from ~19 to ~29 °C. This overlaps with the calculated temperature range for bivalve samples: ~22 to 26 °C (Figure 6). Changing the estimated δ18Owater value used for these temperature calculations results in meaningful changes. In the extreme case, using the measured wintertime δ18Owater value of -7.8 results in a roughly 10 °C decrease for all calculated temperatures. For context, annual temperatures in Ore Lake surface waters range from frozen (≤ 0°C) to approximately 27 °C, with average June through August temperatures ranging from ~20 to ~27 °C (Cooperative Lakes Monitoring Program, 2019; Supplemental 4).
N | Sample Description | δ 13 C (VPDB) | δ 18 O (VPDB) |
1 | South Pisolite 1 subsample A | -3.6 | -7.2 |
2 | South Pisolite 1 subsample B | -2.9 | -6.6 |
3 | South Pisolite 1 subsample C | -3.2 | -7.3 |
4 | South Pisolite 1 subsample D | -3.8 | -7.5 |
5 | South Pisolite 1 subsample | -2.9 | -7.1 |
6 | North Pisolite 1 subsample A | -3.3 | -7.6 |
7 | North Pisolite 1 subsample B | -3.3 | -8.1 |
8 | North Pisolite 1 subsample C | -3.5 | -7.5 |
9 | North Pisolite 1 subsample D | -3.5 | -8 |
10 | North Pisolite 2 | -2.1 | -7.3 |
11 | South Pisolite 2 | -3.1 | -7.8 |
12 | South Pisolite 3 | -2.9 | -7.7 |
13 | South Pisolite 4 | -3.9 | -7.4 |
14 | South Pisolite 5 | -3.3 | -7 |
15 | South Pisolite 6 | -3.3 | -8 |
16 | South Pisolite 7 | -3.5 | -8.3 |
17 | South Pisolite 8 | -2.6 | -7 |
18 | South Pisolite 9 | -1 .1 | -6.3 |
19 | South Pisolite 10 | -1 .7 | -6.7 |
20 | South Pisolite 11 subsample A | -3.2 | -7.9 |
21 | South Pisolite 11 subsample B | -3.2 | -8.2 |
22 | South Pisolite 11 subsample C | -2.6 | -7.6 |
23 | South Pisolite 11 subsample D | -2.7 | -7.7 |
24 | South Pisolite 12 subsample A | -3.2 | -7.8 |
25 | South Pisolite 12 subsample B | -3 | -8.1 |
26 | South Pisolite 12 subsample C | -2.4 | -7.8 |
27 | South Pisolite 12 subsample D | -2.9 | -8 |
28 | South Pisolite 13 | -3.1 | -7.7 |
29 | South Pisolite 14 | -3.2 | -7.8 |
30 | Bivalve 1 - Quagga Mussel | -9.1 | -6.4 |
31 | Bivalve 2 - Asian Clam | -8.4 | -7 |
32 | Bivalve 3 - Quagga Mussel | -5.1 | -7.2 |
33 | Bivalve 4 - Quagga Mussel | -9.1 | -6.5 |
Figure 6. Standard b oxplots of temperatures calculated from carbonate δ18O values using the mean of Ore Lake δ18Owater values (-5.62 ‰ VSMOW, see text). Boxes describe the first and third quartiles of the data, and the horizontal line indicates the median. Both pisoids and local mollusks (quagga mussels and Asian clams) form in summertime temperatures.
4. Key observations and discussion
4.1. Field observations and pisoid morphology
The range of sizes of pisoids in Ore Lake is likely dependent on both the age of the pisoid and the shape of the central nucleation grain. ‘Younger’ (that is, smaller) pisoids that have gone through fewer cycles of summer precipitation often display different morphologies than ‘older’ pisoids, which have a thicker (> 5 mm) build-up of carbonate due to more (and perhaps multiple seasons of) precipitation. ‘Younger’ pisoids may display a shape and size that reflects a gastropod shell nucleus (asymmetric) or may reflect a smaller grained nucleus (more symmetric) (Figure 7). ‘Older’ pisoids fairly consistently show a subspherical morphology regardless of nucleus type. Jones & Wilkinson (1978) suggested that turnover of pisoids in Ore Lake is minimal based on an observed ‘flattening’ of their structures, resulting in slightly asymmetrical banding when viewed in cross section. They suggest this occurs due to consistent contact with the substrate on one side of the pisoid, with slightly more precipitation occurring on all other surfaces (see figure 3 in Jones & Wilkinson, 1978). This interpreted lack of turnover has subsequently been cited as evidence of in-place growth of other sub-spherical carbonate structures (Riding, 1983). In contrast, Casanova (1986) found that the spherical to subspherical morphology of lacustrine concentrically laminated carbonate grains (in that case, described as oncoids) did require continuous displacement to result in similar morphology. Casanova (1986) described consistently rounded and mobile grains in the absence of turbulent waters, although the amount of transportation necessary to cause a subspherical morphology differs depending on factors including grain size and precipitation rate. We suggest that the subspherical geometry of Ore Lake pisoids is also due, at least in part, to growth during transport through the lake. This idea is supported by the existence of large shallow flats throughout the lake that would presumably provide ample area above storm wave base. Pisoid movement during growth is also consistent with field observations of complete pisoids along the lake’s northern and eastern shallows (Figure 1), as well as buildup of a spit at the southern outflow made primarily of pisoid grains (Figure 4).
Figure 7. Gastropod shell grains in various stages of being coated by calcite precipitation.
Water flow through Ore Lake into its southern outlet is considerable. United States Geological Survey (USGS) stream gauge measurements from 2015 show that the streamflow at the southern end of the lake ranges from 12.3 ft3/s to 30.1 ft3/s, with moderate suspended debris recorded at the gauge location during high flow times (USGS, 2015). The majority of pisoids observed on the spit are complete, many of which are > 3 cm. Pisoid fragments and coarse sand is also present, along with very common disarticulated Corbicula sp. and Dreissena sp. shells. This deposition of complete and fragmented pisoids over a ~600 m2 subaerially exposed area shows that transportation certainly takes place, and also that higher-energy storm events play some role in their movement. The only question is whether precipitation and growth occurs at times during this transport.
Pisoid growth during transport is further supported by the generally symmetrical internal laminae and overall shapes of individual pisoids we observed. This is in contrast to the argument by Jones & Wilkinson (1978) for largely immobile pisoids based on observation of consistent asymmetrical shapes, with one side flattened and the opposite side convex, which is visible both on the outermost layer (surface) and within internal laminae. However, we did not find in our samples from Ore Lake that most pisoids today have the distinctly asymmetrical structure they describe. Instead, we observed that only ~29% of pisoid samples (20 out of 70 samples) display any notably flattened bases, where we define a flattened pisoid as having an asymmetrical geometry with one convex surface opposing a more planar surface. This suggests that most pisoids rotate sufficiently for approximately symmetrical growth. Symmetric internal laminae of most pisoids further supports this conclusion (e.g., Figure 3).
Even the minority of pisoids that do have a flatter side may have experienced transport during growth. As noted by Jones & Wilkinson (1978), the question of how cyanobacteria continue photosynthesis on the surface of the pisoid that remains in contact with the substrate – in which carbonate is precipitated in darkness to create a flattened base – remains largely unanswered. Jones & Wilkinson (1978) suggest several possibilities: a) bacterial tolerance to dim light conditions, b) photosynthesis continuing via reflected light, and/or c) the transfer of nutrients via interconnected filaments. Indeed, Braissant et al. (2003) showed that dormant cyanobacterial filaments can trigger carbonate precipitation via extracellular polymeric substances and amino acids in the absence of living cyanobacteria. This may occur in Ore Lake, but it is also possible that these occasional flat sides are instead simply consistent with turnover and transportation of pisoids. Certainly, if transport is occasional and irregular, it would be expected that non-symmetrical precipitation would occur as precipitation slows on the buried side. Given that most pisoids are relatively symmetrical, regular transport of most grains likely occurred. This is further supported by thin section observations described in the below section.
4.2. Thin section and microstructure interpretations
Banding, including concentric banding, within carbonate structures is common and often interpreted as indicating seasonal or annual cyclicity (Dahanayake, 1983; Cassanova, 1986), varying biotic/abiotic influences (Richter & Sedat, 1983), and/or variable precipitation rates (Noiriel et al., 2012). Pisoids in Ore Lake display concentric banding of both porous and dense laminae that were suggested by Jones & Wilkinson (1978) to represent photosynthesis-induced precipitation (rapid and porous) that forms during the summer, and abiotic precipitation in the fall and winter (slow and relatively dense). This interpretation is based on numerous cyanobacterial filaments seen within the porous layers and the lack thereof in the denser layers. We do agree that pisoid thin sections reveal that the more porous layers seem to include more apparent cyanobacterial structures. However, instead of wintertime precipitation, we suggest that the change in the porosity of lamina within Ore Lake pisoids is a result of a changing calcite precipitation rate throughout the spring and summer seasons, and that it is therefore likely that all precipitation is influenced by microbially-induced photosynthetic effects.
In general, the porosity of modern microbial carbonates is controlled by the amount of precipitation in and around cyanobacterial filaments (Wu et al., 2021). Wu et al. (2021) found that more rapid precipitation around cyanobacterial filaments can create calcite ‘crusts’ (precipitation in contact with filaments), with the decay of organic matter creating porosity in-between via voids, and eventually ‘mold holes’. In contrast, 'inter-crustal’ precipitation (precipitation in contact with previously formed calcite) occurs when precipitation slows and results in a denser fabric (Wu et al., 2021). This conceptual framework is consistent with Ore Lake pisoid observations in this study. The lighter, more porous layers show more cyanobacterial structures in thin section (Figure 8) and likely result from calcite crust formation. The faster growth of the more porous layer is further supported by the larger (~ 0.1 mm wide) calcite crystals found occasionally within the layer (Figure 8). These are notably less common in the denser laminae and are consistent with more rapid crystal nucleation and growth (Barth & Chafetz, 2015), as is expected in the higher alkalinity conditions of the early spring and summer months (Figure 5). The darker, less porous layers likely result from slower precipitation and associated inter-crustal precipitation.
Importantly, at the thin section scale we observe generally gradual changes from the more porous to more dense layers. For instance, Figure 8B shows a fan-shaped cyanobacterial growth within the matrix of a pisoid sample. The apex of this mass shows colonization within the porous layer, and an outward growth into the dense layer. Such overlapping of laminae shows that growth is relatively continuous across the boundary. It also indicates that microbial precipitation can be ongoing while a denser lamina forms. We therefore interpret both porous and relatively dense layers to be influenced by microbial communities (i.e., both are ‘biotic’ precipitation), with the change in the porosity resulting primarily from a shift in the precipitation rate.
In contrast to these gradual transitions from more porous layers to more dense layers, the transition from a dense layer to a more porous layer is typically more distinct, with no observed features clearly extending across these boundaries. We therefore interpret these seemingly more abrupt shifts as a temporary cessation of precipitation. In summary, the gradual shift from porous (light) to dense (dark) laminae in Ore Lake pisoids likely represents a slowing precipitation rate as carbonate saturation in the lake water, and the amount of photosynthetic activity decreasing throughout the summer, until the end of precipitation in fall. A new more porous layer rapidly precipitates during the following spring, coinciding with revived photosynthetic activity. This model is further supported by the isotopic results, as discussed in the next section.
Figure 8. Thin section images of a pisoid at 40x magnification. Slides are impregnated with blue dye indicating pore space. A: initial layering nucleating on a gastropod shell. B: Algal filaments are observed to be growing across an apparent light/dark boundary, implying continuous growth.
4.3. Stable isotope interpretations
4.3.1. Carbon isotopes
Carbon isotope (δ13C) values show large variability across sample types, with mollusks as isotopically light as -9.1 ‰ VPDB, and pisoids as isotopically heavy as -1.1 ‰ VPDB (Figure 9). The average pisoid δ13C value of -3 ‰ is markedly more positive than all mollusk values, with three out of the four mollusk shells measured having δ13C values lighter than -8 ‰. This large difference in δ13C values reflects important differences in how carbon is incorporated into these carbonates. Importantly, bivalve shell δ13C is thought to typically precipitate within a few ‰ of ambient dissolved inorganic carbon (DIC; McConnaughey & Gillikin, 2008). Although our study lacks water DIC δ13C measurements, the presumed approximate δ13C equilibrium of bivalve shells enables their use as a proxy for lake water DIC δ13C. Therefore, the positive shift in pisoid δ13C of as much as ~6 ‰, relative to mollusk δ13C, indicates a pisoid-specific carbon isotope fractionation produced during their formation.
Figure 9. Carbon and oxygen stable isotope data for all samples. No difference was observed between pisoids collected at or near the southern spit deposit (black circles) and those collected elsewhere (grey circles). Bivalve values (green squares) are overlapped by pisoid δ18O values entirely, but have very different δ13C values likely due to photosynthetic effects. Error bars (~0.1 ‰) are smaller than points.
The significant δ13C variability across pisoid samples of ~3 ‰ could have many causes. As shown in Figure 10B, δ13C variability within single pisoid is smaller, averaging ~0.6 ‰. This minor variability could plausibly in-part be due to variable amounts of organic decomposition within the pisoids. The larger δ13C range observed across samples is likely to be related to highly localized effects within the lake. These could include the amount of photosynthetic activity, variation in the rate of carbonate precipitation, and/or perhaps spatial differences in alkalinity (sensu Chagas et al., 2016). Importantly, despite this inherent variability, none of the pisoid measurements overlap with shell values. At the very least this several permil difference in δ13C highlights that the two carbonate types are precipitated in ways that fractionate carbon isotopes quite differently.
Recent work has shown that microbialite δ13C values can reflect different factors in different fresh waters, including abiotic lake DIC values (Beeler et al., 2020), sediment type and microbial diversity (Ingalls et al., 2020), mixing of water sources (e.g., thermal and fresh water; Lencina et al., 2023), and carbonate dissolution paired with photosynthesis (Wang et al., 2022). The particular δ13C value of a given microbialite reflects a combination of lake water chemistry (especially Ca/Alk ratios) and biological effects such as photosynthesis (Chagas et al., 2016; McConnaughey, 1989).
Our somewhat limited view of the Ore Lake carbon system (lacking direct lake DIC measurements) inhibits our ability to attribute all the carbonate δ13C variations we observed to specific factors. However, when interpreting the bivalve δ13C data as an approximation of Ore Lake DIC δ13C, we observe a large positive fractionation that is consistent with photosynthetic effects. Preferential incorporation of 12C in organic material is expected during photosynthesis (e.g., McConnaughey, 1989), thereby resulting in higher δ13C values in carbonate formed in association with cyanobacteria (in this case carbonates formed in the pisoids). This interpretation supports our assertion that pisoid growth does not occur abiotically in significant amounts, as all pisoid δ13C measurements are consistent with photosynthetic processes. Similar magnitudes of positive δ13C enrichment relative to lake DIC have been observed in other examples of studies photosynthetic microbial carbonate formation (e.g., Cangemi et al., 2016). These data are also consistent with regular transport, in that precipitation is likely to have occurred primarily on light-exposed sides. Indeed, the lack of differentiation between isotopic values (both δ13C and δ18O) for pisoids collected in different parts of the lake (Figure 9) further suggests that grains are transported during growth, as opposed to forming in discrete, stationary microenvironments.
Figure 10. Higher resolution stable isotope data taken across 4-5 layers from four pisoid samples. A: δ18O data, with conversion to temperature as described in the text. Whilst all temperatures imply warm season precipitation, minor fluctuations may indicate inter-annual variability. B: δ13C data that also suggests inter-annual variability, particularly in the largest sample (SP1).
4.3.2. Oxygen isotopes, temperature, and timing of formation
In contrast to the carbon isotope data, δ18O values for Ore Lake mollusks and pisoids conform, with mollusk data (-7.2 to -6.4 ‰ VPDB) fitting entirely within the pisoid range (-8.3 to -6.3 ‰ VPDB). Although the average shell aragonite δ18O value (-6.8 ± 0.3 ‰) is more positive than the average pisoid calcite δ18O value (-7.5 ± 0.5 ‰), this discrepancy can be largely explained by mineralogical differences. In 25°C water for example, calcite δ18O is expected to be approximately 0.5 ‰ lighter than aragonite (Grossman & Ku, 1986; Kim & O’Neil, 1997). If we make the reasonable assumption that mollusk values reflect approximate oxygen isotopic equilibrium (Wannamaker et al., 2006), then the pisoids must also reflect equilibrium conditions. These data further imply that shell and pisoid carbonate growth occur under very similar temperatures and δ18Owater conditions, and therefore at similar times of year (Figure 6).
Indeed, temperatures derived from pisoid and lake water δ18O values indicate: 1) that carbonate precipitation occurs primarily within the summer months; and 2) within ~3 m of the surface. 85% of the estimated temperatures from pisoid δ18O indicate waters in excess of 22° C, and all data exceed 19 °C (Figure 10A). These warm temperatures are consistent with expected surface water temperatures for an inland lake in Michigan during the summer, and align with available Ore Lake temperature data from May through September (Supplemental 4). Although winter formation of carbonate in freshwater lakes has been directly observed (e.g., Coxon & Coxon, 1994), and has been suggested as occurring in Ore Lake (Jones & Wilkinson, 1978), our data show no evidence for cold water precipitation.
The lack of major δ18O variability within and between separate pisoids also strongly supports formation solely during the warm season. A small amount of apparent temperature seasonality within individual grains (2 – 5 °C) is recorded in our higher resolution sampling of four pisoids (Figure 10A; if we attribute 100% of their δ18O variability to temperature change). In reality, an amount of this variation comes from δ18Owater variability, and so ambient temperatures during porous and dense layer precipitation are remarkably constant for a given pisoid. This is consistent with growth across multiple summers and cessation during colder periods, as we suggest above. And while our warm calculated temperatures should be considered estimates, calculations using a more negative δ18Owater value than measured at the lake in summer and fall within this study, result in non-sensical temperatures. For example, using our wintertime δ18Owater measurement (-7.8 ‰ VSMOW) for temperature calculation with pisoid δ18O values results in an average of ~14 °C, which is much warmer than actual wintertime conditions (~0 °C; Cooperative Lakes Monitoring Program, 2019). Ultimately, the lack of any more positive δ18O outliers (indicating colder temperatures) strongly suggests that very little precipitation occurs during the winter.
To define the timing of pisoid formation in Ore Lake more precisely than simply during the warm season, we can also consider the seasonal alkalinity cycles that are linked to carbonate precipitation in the lake. Historical data from Ore Lake (Fusilier, 1987, 1999) shows an increase in alkalinity at the beginning of the spring season, and a decline during the summer months (from 242 to 169 ppm). A decrease of alkalinity in freshwater lakes generally is often due to carbonate precipitation through dissolved CO2 loss to the atmosphere and photosynthetic processes by aquatic organisms (López-García et al., 2005). Carbonate saturation then begins to increase within the lake water during the fall and winter months, as photosynthetic organisms decrease and winter stratification begins. Müller et al. (2003) found that the total loss of inorganic carbon within lakes with a high dissolved mineral content is directly proportional to the amount of calcite precipitated, further connecting a decrease in alkalinity during the summer months to increased calcite precipitation. Considering both the known cyclical nature of alkalinity within inland lakes, and the 18O results, we suggest that the timing of formation of pisoids in Ore Lake is from late spring (late May) through the summer, and perhaps through and into September. Because of the carbonate and bicarbonate depletion during the summer, we posit that the lower temperatures observed (~19 – 23 °C) may represent late spring precipitation when alkalinity first declines.
4.4 Proposed model of pisoid formation in Ore Lake
Below we suggest a model for Ore Lake pisoid formation that is consistent with our data (Figure 11):
- During fall and winter stratification, lake water alkalinity and carbonate saturation rebound from the summer minima, reaching maximum by spring (as in Figure 5).
- As the temperature rises, cyanobacteria begin to populate on pisoids, shells, grains, and other debris within shallow waters, likely on the wide topographic bench on the eastern side of Ore Lake. Carbonate saturation at this time is at or near maximum. Following this, carbonate precipitation occurs readily and more rapidly. This results in a porous layer due to calcite forming crusts around cyanobacterial filaments, but little inter-crustal precipitation occurs. This rapid precipitation also results in the larger calcite crystals observed within this layer.
- As cyanobacteria continue to increase in population, the saturation decreases as carbonate continues to precipitate, with continued growth on pisoids being increasingly dependent on photosynthetic effects in the micro-environment immediately around the grain. An overall decrease in carbonate saturation thus decreases the precipitation rate of calcite crusts around cyanobacterial filaments. This slower precipitation rate allows more time for inter-crustal precipitation to occur, beginning the transition of from the formation of porous layers to the formation of dense layers.
- By the end of summer, some precipitation still occurs, but it has been significantly reduced due to both carbonate saturation and the amount of photosynthesis beginning to decrease towards autumn. Any precipitation occurring is likely inter-crustal, which continues to create the dense layers of the pisoid. By the autumn and winter, many cyanobacterial filaments that are not encrusted in calcite begin to decay, which results in an increase in porosity.
- Throughout the year, the flow in Ore Lake and storm events cause pisoids to be relatively consistently transported throughout the lake. The rate of this is dependent on both the size of pisoid, as well as flow rate and energy within the lake basin. During spring and summer, this transportation facilitates the precipitation on most sides of most pisoids, resulting in largely symmetric and sub-spherical morphologies. Occasionally, pisoids are not transported due to their size or local effects of obstructions, creating an asymmetric layer.
- This cycle repeats into the next spring, where the porous layer is deposited on the previous year’s dense layer, creating an abrupt contact within the microstructure.
4.5. Ore Lake pisoids in context
Laminated grains with similar morphologies have been described across the geological record (e.g., Dunham, 1969; Simonson & Jarvis, 1990; James et al., 2005; Robins et al., 2015; Chafetz et al., 2018; Laita et al., 2020). The depositional environment of these grains has been interpreted in very different ways, from vadose precipitation (Dunham, 1969) to lagoonal evaporative precipitation (Simonson & Jarvis, 1993), to near-freezing ocean precipitation (James et al., 2005). Certainly, the specific details of the environment of formation in Ore Lake described here differ from many grains with similar morphology. However, our observations provide useful data from multiple perspectives in a modern freshwater setting. For example, our oxygen isotope data show that pisoid structures can form in approximate isotopic equilibrium with ambient waters. Indeed, it is possible that similar unaltered grains in the geological record (while rare) would accurately record past water and temperature conditions. These data also reveal that wintertime precipitation is likely insignificant in Ore Lake and therefore large temperature shifts are not required for concentric banding to form, as local biological and chemical shifts can induce these changes under consistently warm conditions. Simultaneously, our carbon isotope data strongly suggest that Ore Lake pisoid formation is facilitated by microbial photosynthetic processes. This has been similarly reported in other cases (Barth & Chafetz, 2015; Mors et al., 2022), and concentric precipitates likely form in many other freshwater settings, given the general abundance of microbial carbonate precipitation (e.g., Benzerara et al., 2014). The Ore Lake depositional environment can therefore serve as a key analogue and point of comparison for explaining the origin of other large concentric grains in the geological rock record.
Figure 11. A model of pisoid formation described in the text. Briefly, rapid precipitation occurs in late spring and early summer, with slower precipitation continuing throughout the warm season. Pisoid shapes imply that movement occurs throughout this time. Carbonate precipitation then ceases during the cold months.
Acknowledgements
We thank Lora Wingate, Kacey Lohmann, Suzanne Ankerstjerne, and Phoebe Aron for carrying out the stable isotope analyses. Tara Kneeshaw helped supply tools for water chemistry analyses. Funding was provided by the undergraduate Kindschi Fellowship Program at Grand Valley State University.
Authors Contribution
R.L. and I.W. contributed to all aspects of this research paper, including conceptualization, methodology, data collection and analysis, writing and editing of the manuscript, and visualization of the results. Both authors have read and approved the final version of the paper.
Data availability
All quantitative data related to this study are in the manu-script or in the provided supplement as a .xlsx file.
Conflict of interest
We have no conflict of interest to disclose.
References
Andrews, J.E. (2006). Palaeoclimatic records from stable isotopes in riverine tufas: Synthesis and review. Earth-Science Reviews, 75(1-4), 85-104. https://doi.org/10.1016/j.earscirev.2005.08.002
Andrews, S., & Trewin, N. (2014). Paleoenvironmental significance of lacustrine stromatolite forms from the Middle Old Red Sandstone of the Orcadian Basin. Geological Magazine, 151(3), 414-429. https://doi.org/10.1017/S0016756813000290
Barth, J. A., & Chafetz, H. S. (2015). Cool water geyser travertine: crystal Geyser, Utah, USA. Sedimentology, 62(3), 607-620. https://doi.org/10.1111/sed.12158
Beeler, S., Gomez, F., & Bradley, A. (2020). Controls of extreme isotopic enrichment in modern microbialites and associated abiogenic carbonates. Geochimica et Cosmochimica Acta, 269, 136-149. https://doi.org/10.1016/j.gca.2019.10.022.
Benzerara, K., Skouri-Panet, F., Li, J., Férard, C., Gugger, M., Laurent, T., Couradear, E., Ragon, M., Cosmidis, J., Menguy, N., Margaret-Oliver, I., Tavera, R., Lopex-Garcia, P., & Moreira, D. (2014). Intracellular Ca-carbonate biomineralization is widespread in cyanobacteria. Proceedings of the National Academy of Sciences, 111(30), 10933-10938. https://doi.org/10.1073/pnas.1403510111
Binkley, K.L., Wilkinson, B.H., & Owen, R.M. (1980). Vadose beachrock cementation along a southeastern Michigan marl lake. Journal of Sedimentary Petrology, 50(3), 953-962. https://doi.org/10.1306/212F7B30-2B24-11D7-8648000102C1865D
Braissant, O., Cailleau, G., Dupraz, C., & Verrecchia, E. P. (2003). Bacterially induced mineralization of calcium carbonate in terrestrial environments: The role of exopolysaccharides and amino acids. Journal of Sedimentary Research, 73(3), 485-490. https://doi.org/10.1306/111302730485
Braissant, O., Decho, A.W., Dupraz, C., Glunk, C., Przekop, K.M., & Visscher, P.T. (2007). Exopolymeric substances of sulfate-reducing bacteria: Interactions with calcium at alkaline pH and implication of formation of carbonate minerals. Geobiology, 5(4), 401-411. http://doi.org/10.1111/j.1472-4669.2007.00117
Cangemi, M., Censi, P., Reimer, A., D’Alessandro, W., Hause-Reitner, D., Madonia, P., Oliveri, Y., Pecoraino, G., & Reitner, J. (2016). Carbonate precipitation in the alkaline lake Specchio di Venere and the possible role of microbial mats. Applied Geochemistry, 67, 168-176. https://doi.org/10.1016/j.apgeochem.2016.02.012
Casanova, J. (1986). East African Rift stromatolites. Geological Society, London, Special Publications, 25, 201-210. https://doi.org/10.1144/GSL.SP.1986.025.01.17
Chafetz, H., Barth, J., Cook, M., Guo, X., & Zhou, J. (2018). Origins of carbonate spherulites: Implications for Brazilian Aptian pre-salt reservoir. Sedimentary Geology, 365, 21–33. https://doi.org/10.1016/j.sedgeo.2017.12.024
Chafetz, H. S., & Meredith, J. C. (1983). Recent travertine pisoliths (Pisoids) from southeastern Idaho, U.S.A. In T. M. Peryt (eds.), Coated Grains, 450–455. https://doi.org/10.1007/978-3-642-68869-0_39
Chagas, A. A., Webb, G. E., Burne, R. V., & Southam, G. (2016). Modern lacustrine microbialites: towards a synthesis of aqueous and carbonate geochemistry and mineralogy. Earth-Science Reviews, 162, 338-363. https://doi.org/10.1016/j.earscirev.2016.09.012
Cooperative Lakes Monitoring Program (2019). Data Report for Ore Lake, Livingston County. https://micorps.net/lake-monitoring/clmp-documents/
Coxon, C.E., & Coxon, P. (1994). Carbonate deposition in turloughs (seasonal lakes) on the western limestone lowlands of Ireland. Irish Geography, 27(1), 14-27. https://doi.org/10.1080/00750779409478695
Dahanayake, K. (1977). Classification of oncoids from the Upper Jurassic carbonates of the French Jura. Sedimentary Geology, 18(4), 337-353. https://doi.org/10.1016/0037-0738(77)90058-6
Dahanayake, K. (1983). Depositional Environments of Some Upper Jurassic Oncoids. In: Peryt, T.M. (eds) Coated Grains 377-385. https://doi.org/10.1007/978-3-642-68869-0_32
Dunham, R.J. (1969). Vadose pisolite in the Capitan Reef (Permian), New Mexico and Texas. In: Friedman, G.M. Depositional Environments in Carbonate Rocks. https://doi.org/10.2110/pec.69.03.0182
Esteban, M., & Pray, L. C. (1983). Pisoids and pisolite facies (Permian), Guadalupe Mountains, New Mexico and West Texas. In: Peryt, T.M. (eds) Coated Grains. https://doi.org/10.1007/978-3-642-68869-0_43
Folk, R.L., & Chafetz, H.S. (1983). Pisoliths (pisoids) in Quaternary travertines of Tivoli, Italy. In: Peryt, T.M. (eds) Coated Grains. 474-487. https://doi.org/10.1007/978-3-642-68869-0_41
Fusilier, W.E., (1987). A water quality study of Ore Lake: located in Hamburg and Green Oak townships, Livingston County, Michigan. Ore Lake Preservation Association.
Fusilier, W.E., (1999). Ore Lake: A water quality re-study of the lake and its tributaries. Ore Lake Preservation Association.
Grossman, E.L., & Ku, T. (1986). Oxygen and carbon isotope fractionation in biogenic aragonite: Temperature effects. Chemical Geology: Isotope Geoscience Section, 59, 59-74. https://doi.org/10.1016/0168-9622(86)90057-6
Ingalls, M., Frantz, C., Snell, K., & Trower, E. (2020). Carbonate facies-specific stable isotope data record climate, hydrology, and microbial communities in Great Salt Lake, UT. Geobiology, 18, 566-593. https://doi.org/10.1111/gbi.12386
Irion, G., & Müller, G. (1968). Mineralogy, petrology and chemical composition of some calcareous tufa from the Schwäbische Alb, Germany. In: Müller, G., Friedman, G.M. (eds) Recent Developments in Carbonate Sedimentology in Central Europe. 157-171 https://doi.org/10.1007/978-3-642-88052-0_19
James, N. P., Narbonne, G. M., Dalrymple, R. W., & Kyser, T. K. (2005). Glendonites in Neoproterozoic low-latitude, interglacial, sedimentary rocks, northwest Canada: Insights into the Cryogenian ocean and Precambrian cold-water carbonates. Geology, 33(1), 9. https://doi.org/10.1130/G20938.1
Jones, F. G., & Wilkinson, B. H. (1978). Structure and growth of lacustrine pisoliths from recent Michigan marl lakes. Journal of Sedimentary Research, 48(4), 1103-1110. https://doi.org/10.1306/212F75FE-2B24-11D7-8648000102C1865D
Kim, S., & O’Neil, J.R(1997). Equilibrium and nonequlibrium oxygen isotope effects in synthetic carbonates. Geochimica et Cosmochimica Acta, 61(16), 3461-3475. https://doi.org/10.1016/S0016-7037(97)00169-5
Laita, E., Bauluz, B., Aurell, M., Badenas, B., Canudo, J. I., & Yuste, A. (2020). A change from warm/humid to cold/dry climate conditions recorded in lower Barremian clay-dominated continental successions from the SE Iberian Chain (NE Spain). Sedimentary geology, 403, 105673. https://doi.org/10.1016/j.sedgeo.2020.105673
Lencina, A., Soria, M., Colla, M., Cury, L., Farías, E., & Gomez, F. (2023). In situ growth of modern oncoids from Salado river, Salar de la Laguna Verde Complex, Argentina. Sedimentary Geology, 451. https://doi.org/10.1016/j.sedgeo.2023.106396.
López-García, P., Kazmierczak, J., Benzerara, K., Kempe, S., Guyot, F., & Moriera, D. (2005). Bacterial diversity and carbonate precipitation in the giant microbialites from the highly alkaline Lake Van, Turkey. Extremophiles, 9, 263-274. https://doi.org/10.1007/s00792-005-0457-0
Maliva, R. G., Missimer, T. M., Leo, K. C., Statom, R. A., Dupraz, C., Lynn, M., & Dickson, J. A. D. (2000). Unusual calcite stromatolites and pisoids from a landfill leachate collection system. Geology, 28(10), 931-934. https://doi.org/10.1130/0091-7613(2000)28%3C931:UCSAPF%3E2.0.CO;2
McConnaughey, T. (1989). 13C and 18O isotopic disequilibrium in biological carbonates: II. In vitro simulation of kinetic isotope effects. Geochimica et Cosmochimica Acta, 53, 163-171. https://doi.org/10.1016/0016-7037(89)90283-4
McConnaughey, T. A., & Gillikin, D. P. (2008). Carbon isotopes in mollusk shell carbonates. Geo-Marine Letters, 28(5–6), 287–299. https://doi.org/10.1007/s00367-008-0116-4
Merz, M.U.E. The biology of carbonate precipitation by cyanobacteria. (1992). Facies, 26, 81-101. https://doi.org/10.1007/BF02539795
Merz-Preiß, M., & Riding, R. (1999). Cyanobacterial Tufa Calcification in Two Freshwater Streams: Ambient Environment, Chemical Thresholds and Biological Processes. Sedimentary Geology, 12.6(1-4), 103-124. https://doi.org/10.1016/S0037-0738(99)00035-4
Monty, C.L., & Mas, J.R. (1981). Lower Cretaceous (Wealdian) Blue-Green Algal Deposits of the Province of Valencia, Eastern Spain. In: Monty, C. (eds) Phanerozoic Stromatolites. 85-120. https://doi.org/10.1007/978-3-642-67913-1_9
Mors, R. A., Gomez, F. J., Astini, R. A., Celestian, A. J., & Corsetti, F. A. (2022). Assessing the origin of pisoids within a travertine system in the border of Puna Plateau, Argentina. Sedimentology, 69(3), 1252-1275. https://doi.org/10.1111/sed.12946
Müller, B., Meyer, J. S., & Gächter, R. (2016). Alkalinity regulation in calcium carbonate-buffered lakes: Alkalinity regulation in calcium carbonate-buffered lakes. Limnology and Oceanography, 61(1), 341–352. https://doi.org/10.1002/lno.10213
Müller, B., Wang, Y., Dittrich, M., & Wehrli, B. (2003). Influence of organic carbon decomposition on calcite dissolution in surficial sediments of a freshwater lake. Water Research, 37(18), 4524-4532. https://doi.org/10.1016/S0043-1354(03)00381-6
Noiriel, C., Steefel, C., Yang, L., & Ajo-Franklin, J. (2012). Upscaling calcium carbonate precipitation rates from pore to continuum scale. Chemical Geology, 318(60-74). https://doi.org/10.1016/j.chemgeo.2012.05.014
Otsuki, A., & Wetzel, R. G. (1974). Calcium and total alkalinity budgets and calcium carbonate precipitation of a small hard-water lake. Archiv für Hydrobiologie, 14-30. https://doi.org/10.1127/archiv-hydrobiol/73/1974/14
Parker, A., & Kohlhepp, G. (2017). Michigan inland lake harmful algal bloom toxin study. Michigan Department of Environmental Quality Work Plan and Quality Assurance Project Plan. 1-17. https://www.michigan.gov/egle/-/media/Project/Websites/egle/Documents/Programs/WRD/SWAS/HAB/habs-qapp-2017.pdf?rev=a4dfc6fb83c74f7b917931d71bfddd9e&hash=2E7EFC298AFE93E1EA53D306C13CCF3C
Pedley, H. M. (1990). Classification and environmental models of cool freshwater tufas. Sedimentary Geology, 68(1-2), 143-154. https://doi.org/10.1016/0037-0738(90)90124-C
Pollock, J.B. (1919). Blue-Green Algae as Agents in the Deposition of Marl in Michigan Lakes. Michigan Academy of Science, Arts, and Letters 20, 247-260.
Reid, R. P., Visscher, P. T., Decho, A. W., Stolz, J. F., Bebout, B. M., Dupraz, C., Macintyre, I.G., Paerl, H.W., Picknewy, J.L., Prufert-Bebout, L., Steppe, T.F. & DesMarais, D. J. (2000). The role of microbes in accretion, lamination and early lithification of modern marine stromatolites. Nature, 406(6799), 989-992. https://doi.org/10.1038/35023158
Richter, D.K., & Sedat, R. (1983). Brackish-water oncoids composed of blue-green and red algae from a Pleistocene terrace near Corinth, Greece. In: Peryt, T.M. (eds) Coated Grains. 299-307. https://doi.org/10.1007/978-3-642-68869-0_26
Riding, R. (1983). Cyanoliths (Cyanoids): Oncoids formed by calcified cyanophytes. In: Peryt, T.M. (eds) Coated Grains. 276-283. https://doi.org/10.1007/978-3-642-68869-0_24
Risacher, F., & Eugster, H. P. (1979). Holocene pisoliths and encrustations associated with spring-fed surface pools, Pastos Grandes, Bolivia. Sedimentology, 26(2), 253–270. https://doi.org/10.1111/j.1365-3091.1979.tb00353.x
Rivera, M. J., & Sumner, D. Y. (2014). Unraveling the three-dimensional morphology of Archean microbialites. Journal of Paleontology, 88(4), 719–726. https://doi.org/10.1666/13-084
Robins, C. R., Deurlington, A., Buck, B. J., & Brock-Hon, A. L. (2015). Micromorphology and formation of pedogenic ooids in calcic soils and petrocalcic horizons. Geoderma, 251, 10-23. https://doi.org/10.1016/j.geoderma.2015.03.009
Shiraishi, F., Hanzawa, Y., Asada, J., Cury, L. F., & Bahniuk, A. M. (2022). Microbial influences on tufa deposition in a tropical climate. Sedimentary Geology, 427, 106045. https://doi.org/10.1016/j.sedgeo.2021.106045
Simonson, B. M., & Jarvis, D. G. (1993). Microfabrics of Oolites and Pisolites in the Early Precambrian Carawine Dolomite of Western Australia. In R. Rezak & D. L. Lavoie (eds.), Carbonate Microfabrics 227–237. https://doi.org/10.1007/978-1-4684-9421-1_17
Turchyn, A. V., Bradbury, H. J., Walker, K., & Sun, X. (2021). Controls on the precipitation of carbonate minerals within marine sediments. Frontiers in Earth Science, 9, 618311. https://doi.org/10.3389/feart.2021.618311
United States Geological Survey (USGS), 2015. Surface Water for Michigan: Streamflow Measurements. https://waterdata.usgs.gov/mi/nwis/measurements
Wanamaker Jr, A. D., Kreutz, K. J., Borns Jr, H. W., Introne, D. S., Feindel, S., & Barber, B. J. (2006). An aquaculture-based method for calibrated bivalve isotope paleothermometry. Geochemistry, Geophysics, Geosystems, 7(9). https://doi.org/10.1029/2005GC001189
Wang, W., Li, S., Zhong, J., Slowinski, S., Li, S., Li, C., Su, J., Yi, Y., Dong, K., Xu, S., Van Cappellen, P., & Liu, C. (2022). Carbonate mineral dissolution and photosynthesis-induced precipitation regulate inorganic carbon cycling along the karst river-reservoir continuum, SW China, Journal of Hydrology, 615. https://doi.org/10.1016/j.jhydrol.2022.128621.
Wu, Y. S., Jiang, H. X., Li, Y., & Yu, G. L. (2021). Microfabric features of microbial carbonates: experimental and natural evidence of mold holes and crusts. Journal of Palaeogeography, 10(1), 1-13. https://doi.org/10.1186/s42501-021-00100-5
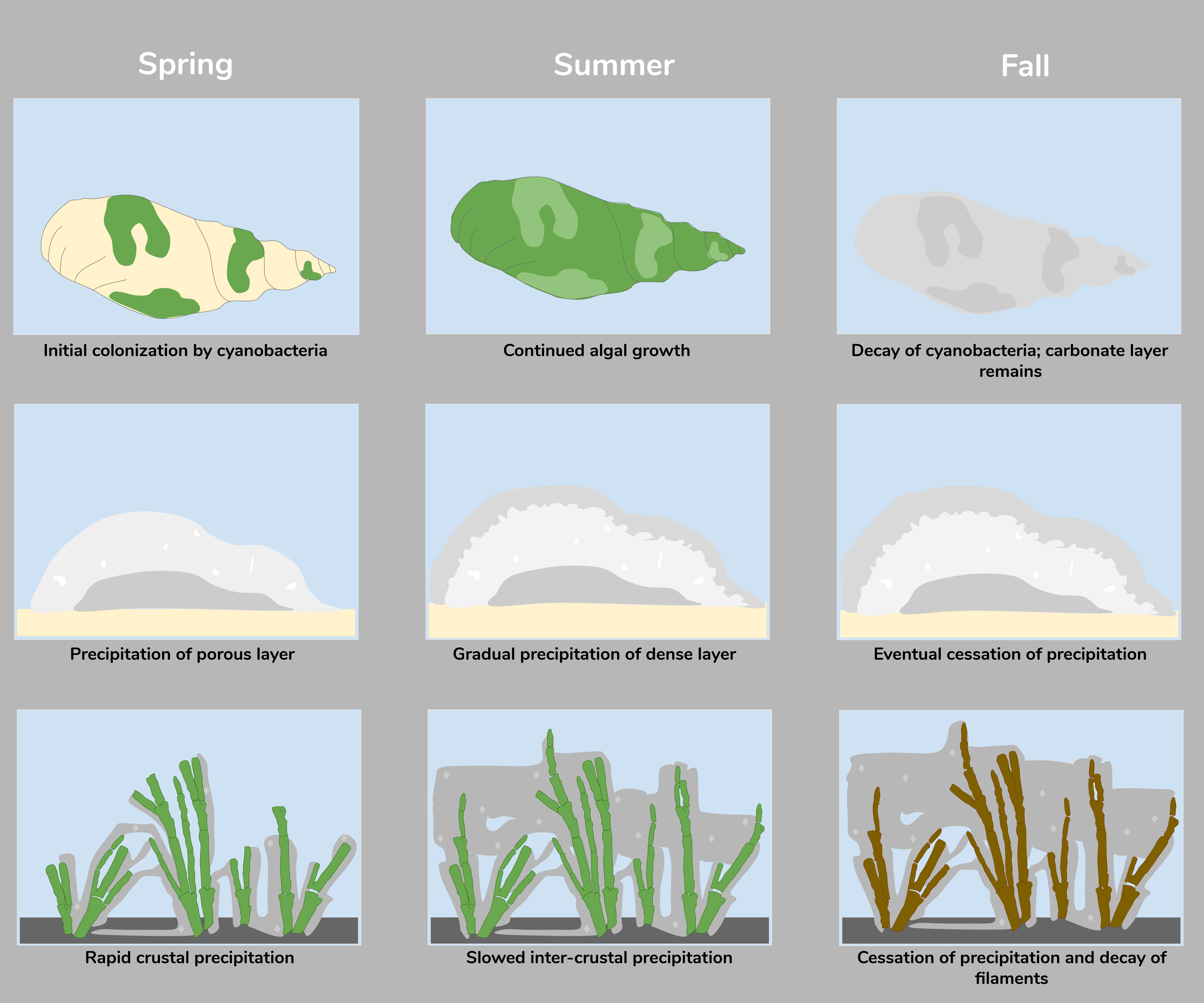
Downloads
How to Cite
License
Some rights reserved 2023 Ryleigh Landstra, Ian Winkelstern

This work is licensed under a Creative Commons Attribution 4.0 International License.